Biochemist studies how ribosomes make proteins
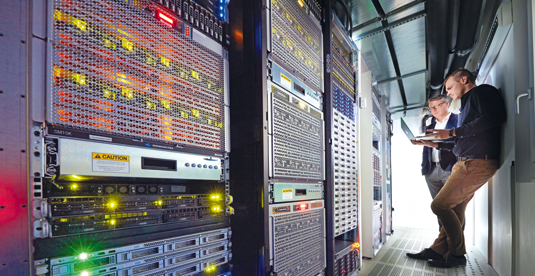
Ribosomes are molecular machines programmed by genetic blueprints, which make proteins by linking amino acids together into linear chains that fold into sequence-dependent shapes. Ludwig Maximilian University biochemist Roland Beckmann studies how they do it.
Roland Beckmann is a basic researcher – in two senses. He studies fundamental biochemical processes that take place in all cell types, and he analyzes the molecular complexes that carry them out in the finest possible detail. When he talks about his findings, he transports the listener into a world where minuscule biological machines form nanoscale production lines that turn out intricately structured macromolecules to order. Their products are in turn responsible for the assembly, packaging and dispatch of myriads of other molecules. These processes are the very stuff of life.
Beckmann, Professor of Biochemistry at LMU's Gene Center, specializes in the investigation of ribosomes, which textbooks refer to as 'the sites of protein synthesis' in cells. This is true as far as it goes, but researchers continue to discover new aspects of the regulation and dynamics of the process, and many of the structural details remain unknown. One thing is clear – protein production in cells is mass production. A single yeast cell may contain up to 200,000 ribosomes, a human liver cell may have up to a million. When one considers that an adult human is made up of over a billion cells, the magnitude of the task of the protein-synthesizing machinery, and its indispensability at every second of our existence, begins to dawn on us. "Ribosomal assembly-lines are constantly on the go," says Beckmann.
How then do cells set about making proteins, the instructions for which are stored in their genetic material? This is the question at the heart of Beckmann's research. As a biochemist, he develops new analytical techniques with which to measure, perturb, monitor and model gene regulatory processes. The goal is to understand biological systems in all their complexity, in particular the dense networks of intermolecular communications that keep cells alive, each one representing a metastable system held together by sensors, signals and interactions. A typical human cell is made up of a surface membrane, and so-called organelles including the nucleus, membrane-bounded compartments and macromolecular complexes, which carry out specific and vital tasks. The nucleus harbors the genetic material – double-stranded DNA packaged into DNA-protein complexes – and controls all cellular functions; mitochondria provide energy, lysosomes dispose of proteins, and ribosomes synthesize proteins.
Deciphering the book of genes
Researchers basically understand how ribosomes use the information encoded in the DNA of the 'genome' to build thousands of different proteins. The genome can be thought of as a collection of blueprints for building the organism. These only make sense if they can be accessed, read and the instructions they contain used to direct the construction of the molecules they specify. Ribosomes are responsible for implementing these plans, which are encoded in defined sequences of DNA subunits called bases. Programmed by the genetic text, ribosomes assemble all the proteins – enzymes that catalyze chemical reactions, components of the cell's internal skeleton, antibodies that recognize pathogens – the organism needs for its growth and survival. And since a protein's function is largely dependent on its shape, ribosomes can be thought of as 3-D printers.
The details of the process are rather more complicated. The base sequence specifying the structure of a given protein is first copied from the appropriate segment of the coding strand of the double-stranded DNA into messenger RNA (mRNA) molecules, which are single-stranded. Ribosomes themselves comprise several 'ribosomal RNAs' and 50-80 proteins and consist of two subunits. The mRNA is fed into the smaller subunit – rather like threading a bicycle chain onto a sprocket wheel – and its base sequence is decoded. The code is both clever and efficient. It is read successively in non-overlapping sets of three bases (known as triplets or codons), and the specific combination of bases in a triplet tells the ribosome which amino acid should be inserted at that position in the protein chain. As DNA and RNA sequences contain four different types of bases, 4×4×4 or 64 different combinations are possible. However, since only 20 distinct amino acids are found in proteins, most amino acids are encoded by more than one codon, while four triplets serve as punctuation signals. Thus the ribosome proceeds from the start signal to a stop signal, reading the code in threes, and capturing and linking up amino acids in the specified sequence.
Proteins can comprise up to several thousand amino acid subunits, and the growing chain is fed into a 10-nanometer (10-8 cm) long exit tunnel in the large subunit of the ribosome, emerging either as an already three-dimensionally folded molecule or as a randomly coiled chain, depending on the protein concerned. "It is a fascinating fact that all organisms, from microbes to humans, possess these machines," says Beckmann. "All living things use basically the same genetic language and the same type of code, which implies that the triplet code was established early in evolution. Over time, however, the ribosomes became more and more complex."
Structural biologists have long known that all ribosomes consist of a large and a small subunit, though these differ somewhat in structure between lower (prokaryotic) and higher (eukaryotic) organisms. But the rapid progress made in analyzing their structures in the past two decades is largely due to the advent of cryo-electron microscopy. "Technology is crucial for us," Beckmann remarks. He became acquainted with the method when he was a postdoc in Günter Blobel's laboratory at Rockefeller University in New York City, although he learned about it not from Blobel (who went on to win a Nobel Prize) but from Joachim Frank, who pioneered the technique and was using it to analyze molecular structures in the state capital, Albany. "Cryo-electron microscopy was a new frontier at the time," Beckmann says, but it would become the basis of his own area of research.
As the prefix suggests, samples for cryo-electron microscopy must first be cooled – or rather flash frozen. Ribosomes or ribosomal complexes, painstakingly isolated from nucleated or bacterial cells, are placed on a thin carbon film deposited on a copper grid and frozen by plunging them into liquid ethane. The nanomachines are instantaneously captured while they are at work and can be subsequently imaged by electron microscopy. Moreover, the micrographs can be interpreted as snapshots of the process of protein synthesis. Because the glass-like solid in which they are immobilized is vitrified ice which (unlike normal ice) contains no crystals, the fragile and complex architecture of the ribosomes is preserved in such detail that the researchers can reconstruct the sequence of steps involved in protein production and view it like a time-lapse movie. "Depending on the protein and organism involved, synthesis can take anywhere from a few seconds to several minutes," says Beckmann. "We want to observe the crucial transition stages."
Novel direct electron detectors have revolutionized the practice of cryo-electron microscopy. Researchers call it the 'resolution revolution', because it has greatly enhanced the resolution of the micrographs, to a few tenths of a nanometer. Visitors to Beckmann's department in the Gene Center in Grosshadern can observe not only the preparation of samples in the lab, but join his research staff as they inspect the data being acquired by the electron microscope. Some screens are filled with columns of numbers; some researchers are analyzing the micrographs themselves. It is even possible to switch to what the detectors are "seeing". These "live" pictures are normally shown on the big monitor in the department's kitchen. These provide the unprocessed data that form the basis for the high-resolution two-dimensional images, taken from different angles, which are used to construct three-dimensional views. In fact, the investigators can actually zoom in to study the finer details.
Integration into the cell membrane
Beckmann is particularly interested in one particular component of the cells of higher organisms – the Sec61 complex or translocon, onto which ribosomes can dock. The translocon acts as a conduit for proteins and plays a key role whenever proteins need to be inserted into or transported through a membrane. About one-third of all proteins are either inserted into the cell membrane where they serve as signal receptors, Beckmann explains, or are secreted by the cell, to act as of antibodies or digestive enzymes, for instance. Beckmann's group has used structural analysis to decipher how the so-called ribosome-translocon complex orchestrates the passage of proteins into and through membranes. The Sec61 complex itself forms a molecular channel into which the ribosome directs the growing protein. Beckmann determined the precise structure of this channel last year. The findings revealed that the ribosome binds directly to the translocon on the membrane and feeds the nascent protein into the channel, and either into the membrane or straight through it. In order to understand how the process works, it is necessary to analyze the structure of the whole complex in as many functional states as possible.
For proteins destined to be integrated into membranes or secreted to the outside, the ribosome needs to know the correct destination of each. Günter Blobel discovered that proteins carry a kind of postal code at the front end of the amino-acid sequence, a zip code that can be read by the proteins of the cell's routing system. This short sequence ensures that proteins are piloted to the right location. The idea was initially met with skepticism, but Blobel, one of the "original gangsters" (Beckmann's term) in the field, turned out to be right. The basic principle was found to be generally valid, and functions in the same way in yeasts as in plant and animal cells. And Blobel won the 1999 Nobel Prize for demonstrating it. Beckmann was a postdoc in Blobel's lab at the time and followed the awards ceremony live. "It was as if a tsunami had hit the lab," he recalls.
Ribosome research has experienced a boom in recent years, but many of the organelle's functions remain mysterious. For instance, Beckmann would love to know how the protein factories carry out quality control. Clearly, they are equipped to detect errors in the blueprint (i.e., the mRNA), and can assess whether the growing protein is functional. "There is nothing more damaging for an organism than the accumulation of defective proteins encoded by corrupted mRNAs," Beckmann says, because this can lead to the loss of essential functions. But whenever a ribosome reaches the end of an mRNA without having encountered a termination codon, for instance, it "knows" that the protein is incomplete – because it cannot be released. The stalled ribosome then recruits specialized release factors that detach it from the defective mRNA and its protein product, and ensure that both are degraded.
A class of cellular apps
Beckmann has been studying ribosomes for nearly two decades, and he speaks of these – in molecular terms – huge complexes, with dimensions of up to 35 nm, with something like respect. Ribosomes are not perfect, but they are extremely versatile machines, he says. Only very recently, researchers realized that, in addition to their primary task, they also have many part-time duties, in relation to quality control, for example. It turns out that an array of accessory proteins can bind to the surface of the ribosome to facilitate the recognition and translation of codons in the mRNA, and monitor and promote the growth of its protein products. Each factor is like an app that enables the ribosome to do something amazing, Beckmann says. "For example, they can measure the forces acting on cell components, and estimate amino acid and antibiotic concentrations. The spectrum of specialties is broad and varies from organism to organism."
Beckmann is not only interested in how the molecular machines work. His team is now looking at what happens to the ribosome when it comes off the job. Essentially, the organelle dissociates into its two subunits, which are then ready for the next round of synthesis. "Only recently have we learned that, in eukaryotic cells, the enzyme ABCE1 has a major influence on this step," Beckmann explains. Basically it uses a lever to force the subunits apart, and since ABCE1 contains clusters of bound iron, Beckmann refers to this as 'the iron fist'. Members of his group are now trying to find out how this essential step in protein synthesis works in structural terms. "This sort of work can take months or years," he adds.
It is conceivable that the future may provide us with a completely new picture of the mechanisms involved in protein synthesis. "Very often, in pursuit of one goal, we stumble across something unexpected that we also need to understand," says Beckmann. It looks as if he's unlikely to run out of puzzles for him and his coworkers to solve in the coming years. "At all events," he avers, "ribosomes have a few more surprises in store for us."
Provided by Ludwig Maximilian University of Munich