Theorem unifies superfluids and other weird materials
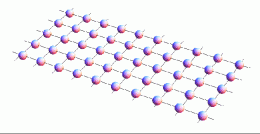
(Phys.org) -- Matter exhibits weird properties at very cold temperatures. Take superfluids, for example: discovered in 1937, they can flow without resistance forever, spookily climbing the walls of a container and dripping onto the floor.
In the past 100 years, 11 Nobel Prizes have been awarded to nearly two dozen people for the discovery or theoretical explanation of such cold materials – superconductors and Bose–Einstein condensates, to name two – yet a unifying theory of these extreme behaviors has eluded theorists.
University of California, Berkeley, physicist Hitoshi Murayama and graduate student Haruki Watanabe have now discovered a commonality among these materials that can be used to predict or even design new materials that will exhibit such unusual behavior. The theory, published online June 8 by the journal Physical Review Letters, applies equally to magnets, crystals, neutron stars and cosmic strings.
“This is a particularly exciting result because it concerns pretty much all areas of physics; not only condensed matter physics, but also astrophysics, atomic, particle and nuclear physics and cosmology,” said Murayama, the MacAdams Professor of Physics at UC Berkeley, a faculty senior scientist at Lawrence Berkeley National Laboratory and director of the Kavli Institute for the Physics and Mathematics of the Universe at the University of Tokyo. “We are putting together all of them into a single theoretical framework.”
The theorem Watanabe and Murayama proved is based on the concept of spontaneous symmetry breaking, a phenomenon that occurs at low temperatures and leads to odd behavior. This produces superconductors, which allow electric currents to flow without resistance; or Bose-Einstein condensates, which have such low energy that every atom is in the same quantum state.
By describing the symmetry breaking in terms of collective behavior in the material – represented by so-called Nambu-Goldstone bosons – Murayama and Watanabe found a simple way to classify materials’ weirdness. Boson is the name given to particles with zero or integer spin, as opposed to fermions, which have half-integer spin.
“Once people tell me what symmetry the system starts with and what symmetry it ends up with, and whether the broken symmetries can be interchanged, I can work out exactly how many bosons there are and if that leads to weird behavior or not,” Murayama said. “We’ve tried it on more than 10 systems, and it works out every single time.”
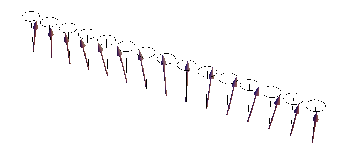
Anthony Leggett of the University of Illinois at Urbana Champaign, who won the 2003 Nobel Prize in Physics for his pioneering work on superfluids, pointed out that “it has long been appreciated that an important consequence of the phenomenon of spontaneously broken symmetry, whether occurring in particle physics or in the physics of condensed matter, is the existence of the long-wavelength collective excitations known as Nambu-Goldstone bosons.
“In their paper, Watanabe and Maruyama have now derived a beautiful general relation … (involving) Nambu Goldstone bosons … (that) reproduces the relevant results for all known cases and gives a simple framework for discussing any currently unknown form of ordering which may be discovered in the future.”
“Surprisingly, the implications of spontaneous symmetry breaking on the low energy spectrum had not been worked out, in general, until the paper by Watanabe and Murayama,” wrote Hirosi Ooguri, a professor of physics and mathematics at Caltech. “I expect that there will be a wide range of applications of this result, from condensed matter physics to cosmology. It is a wonderful piece of work in mathematical physics.”
Discover the latest in science, tech, and space with over 100,000 subscribers who rely on Phys.org for daily insights. Sign up for our free newsletter and get updates on breakthroughs, innovations, and research that matter—daily or weekly.
Symmetry
Symmetry has been a powerful concept in physics for nearly 100 years, allowing scientists to find unifying principles and build theories that describe how elementary particles and forces interact now and in the early universe. The simplest symmetry is rotational symmetry in three dimensions: a sphere, for example, looks the same when you rotate it arbitrarily in any direction. A cylinder, however, has a single rotational symmetry around its axis.
Some interactions are symmetric with respect to time, that is, they look the same whether they proceed forward or backward in time. Others are symmetric if a particle is replaced by its antiparticle.
When symmetry is broken spontaneously, new phenomena occur. Following the Big Bang, the universe cooled until its symmetry was spontaneously broken, leading to a predicted Higgs boson that is now being sought at the Large Hadron Collider in Geneva, Switzerland.
With solids, liquids or gases, symmetry relates to the behavior of the spins of the atoms and electrons. In a ferromagnetic material, such as iron or nickel, the randomness of the electron spins at high temperatures makes the material symmetric in all directions. As the metal cools, however, the electron spins get locked in and force their neighbors to lock into the same direction, so that the magnet has a bulk magnetic field pointing in one direction.
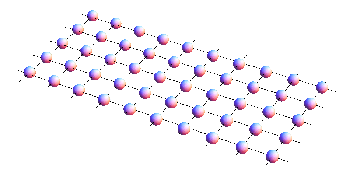
Nambu-Goldstone bosons are coherent collective behavior in a material. Sound waves or phonons, for example, are the collective vibration of atoms in a crystal. Waves of excitation of the electron spin in a crystal are called magnons. During the cooling process of a ferromagnet, two symmetries were spontaneously broken, leaving only one Nambu-Goldstone boson in the material.
In Bose-Einstein condensates, for example, “you start with a thin gas of atoms, cool it to incredibly low temperature — nanokelvins — and once you get to this temperature, atoms tend to stick with each other in strange ways,” Murayama said. “They have this funny vibrational mode that gives you one Nambu-Goldstone boson, and this gas of atoms starts to become superfluid again so it can flow without viscosity forever.”
On the other hand, solid crystals, regardless of their compositions or structures, have three Nambu-Goldstone bosons, equivalent to the three vibrational modes (phonons).
“What this Nambu-Goldstone boson is, how many of them there are and how they behave decide if something becomes a superfluid or not, and how things depend on the temperature,” Murayama added. “All these properties come from how we understand the Nambu-Goldstone boson.”
Yoichiro Nambu shared the 2008 Nobel Prize in Physics, in part, for explaining that in some systems, the number of broken symmetries equals the number of Nambu-Goldstone bosons.
The new theorem expands on Nambu’s ideas to the more general case, Watanabe said, proving that in weird materials, the number of Nambu-Goldstone bosons is actually less than the number of broken symmetries.
“What Nambu showed was true, but only for specialized cases applicable to particle physics,” he said. “Now we have a general explanation for all of physics; no exceptions.”
One characteristic of states with a low Nambu-Goldstone boson number is that very little energy is required to perturb the system. Fluids flow freely in superfluids, and atoms vibrate forever in Bose-Einstein condensates with just a slight nudge.
As a student at the University of Tokyo, Watanabe had proposed a theorem to explain materials’ properties through Nambu-Goldstone bosons, but was unable to prove it until he came to UC Berkeley last year and talked with Murayama. Together, they came up with a proof in two weeks of what they call a unified theory of Nambu-Goldstone bosons.
“Those two weeks were very exciting,” Watanabe said.
More information: Unified Description of Non-Relativistic Nambu–Goldstone bosons, arxiv.org/pdf/1203.0609v2.pdf (PDF submitted to Physical Review Letters)
Journal information: Physical Review Letters
Provided by University of California - Berkeley